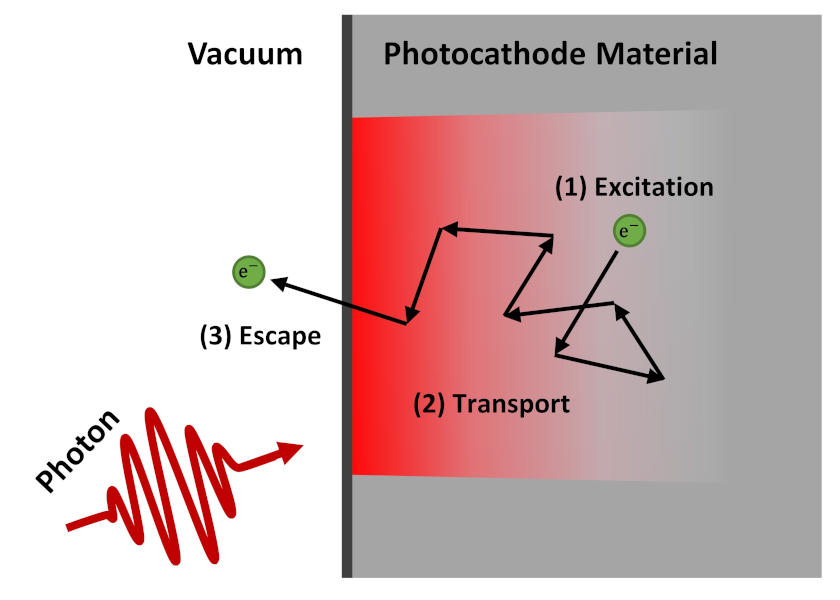
What is a Photocathode?
Photocathodes convert light into electrons by the photoelectric effect. In the photoemission process, photons (light) first penetrate the photocathode material where they can excite (give their energy to) electron-hole pairs. Some energetic electrons can make it to the surface of the photocathode and, if they make it over the surface barrier, can escape into the vacuum completing the emission process. Each of these three steps (excitation, transport, and escape into vacuum) depends strongly on the properties of the photocathode material. In turn, the important performance parameters for photocathode applications also depend strongly on what material is used. Photocathodes can be operated in two modes: reflection mode where the electrons escape from the same side as the illumination source, and transmission mode where they escape from the opposite side.
Types of Photocathodes
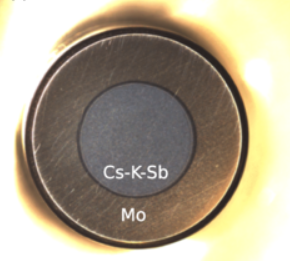
There are several families of materials commonly used as photocathodes, each with its own unique characteristics. Metal photocathodes (such as copper) are typically very stable and survive in harsh conditions such as those found in some RF photoinjectors. However, they also tend to have a lower quantum efficiency than other photocathode materials and a high work function that requires ultraviolet light to be used to drive them. The III-V semiconductors (for example, GaAs) have a long history of use as photocathodes. After activation to negative electron affinity, they benefit from some of the best achievable quantum efficiencies but also require pristine vacuum conditions to avoid degradation. The alkali antimonide family (which, as an example, includes cesium antimonide) holds the record for the highest average current achieved from a photoinjector. This list is by no means all-inclusive and researchers continue to study new materials all the time.
Photo by Martin A. H. Schmeißer / CC BY
Applications of Photocathode Materials
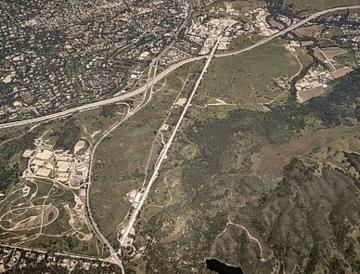
Photoinjectors
Photocathodes are a common choice when scientists need to generate high-brightness electron beams. The LCLS-II x-ray free electron laser at SLAC (pictured here), for example, is driven with a Cs-Te photocathode. In this application, a laser is used to illuminate a spot on the photocathode surface and the emitted electrons are accelerated into a beam with a strong electric field. The piece of equipment used to generate electron beams using a photocathode is called a photoinjector. The properties of the photocathode can place severe limits on the quality of the beam produced by photoinjectors and researchers continue to explore new materials to improve their performance in this application.
Photo by Dicklyon / CC BY
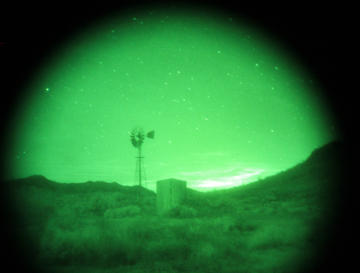
Image Intensifiers
Photocathodes are widely used in image intensifiers which amplify scenes with low levels of light to be visible to the human eye or a camera sensor. In these devices, the photocathode material converts incoming light into electrons, which are then accelerated and amplified using electron multiplier devices such as a micro-channel plate. The amplified electrons are converted back into light to form a visible image using a phosphor screen. Image intensifiers have a wide range of applications including night vision goggles, medical imaging technology, and low-light cameras for industry and scientific research.
Photo by Andrew Loescher / CC BY
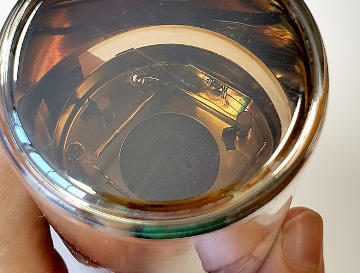
Photomultiplier Tubes
Photocathodes are an essential part of photomultiplier tubes which are used to detect low-level light signals in a variety of applications. These applications can include particle physics, nuclear physics, medical imaging, astronomy, and materials science. In a photomultiplier tube, the photocathode is used to convert light into electrons which are then amplified by a series of "dynodes." These dynodes are coated in a material with a high secondary electron coefficient and each subsequent one is kept at a higher potential than the other. Incoming electrons are multiplied at each stage and the resulting avalanche is captured by an anode at the output to produce a measurable electrical signal.
Photo by Jan Helebrant / CC0
Photocathode Properties and Performance Metrics
To assess the performance of photocathodes, researchers use a variety of metrics that are useful for understanding their suitability for a variety of applications. In this section, we will describe the most commonly used metrics and give definitions for how they are reported in this database. It should be noted that alternative definitions may exist for some metrics, and we don't claim the following to be universally adopted.
Quantum Efficiency (QE)
Quantum efficiency is a measure of how "sensitive" the photocathode is to light. It is defined as the ratio of electrons emitted per the number of incident photons. The number can be calculated from photoemission measurements as \(\text{QE}=1234\cdot I [A] /(\lambda [nm] \cdot P [W])\) with \(I\) as the current from the photocathode, \(P\) the power of the light source, and \(\lambda\) as its wavelength. The quantum efficiency, and in particular the plot of a photocathode's quantum efficiency versus wavelength, is also known as its spectral response.
Mean Transverse Energy (MTE) and Thermal Emittance
Mean transverse energy (MTE) is a measure of the emitted electron's uncorrelated motion in the plane of the cathode's surface. In particle accelerators, it plays a role in setting the brightness of the electron beam. The MTE (commonly measured in meV) is calculated as \(\text{MTE} = E\left[(p_x^2 + p_y^2)/(2m_e)\right]\) where the expectation value \(E[x]\) is taken over the distribution of all electrons emitted from the photocathode. The value \(m_e\) is the electrons' mass and \(p_x\) and \(p_y\) are the components of momenta in the direction of the photocathode's surface (ie \(\hat{z}\) is the surface normal vector). Thermal emittance is a related concept and can be calculated from MTE as \(\varepsilon_{th}\ [\mu\text{m/mm}] = \sqrt{\text{MTE [meV]}/511}\).
Spin Polarization
Some photocathodes can be made to emit electron beams where the quantum mechanical spins of the particles mostly take on the same value. These types of cathodes have important applications in science such as a future electron ion collider which will use polarized electron beams to probe the physics of the nucleus. One way of quantifying the purity of the electrons' spin state is \(P = (N_\uparrow - N_\downarrow)/(N_\uparrow + N_\downarrow)\) where \(N_\uparrow\) is the number that are spin up and \(N_\downarrow\) is the number that are spin down.
Nonlinear Yield
When photocathodes are driven with high-intensity light (such as from an ultrafast laser) the rate of electrons emitted per unit of optical intensity can deviate from its normally linear relationship. This can be interpreted as multiphoton interactions contributing to the emission process (that is, two or more photons acting to excite and emit a single electron). Nonlinear yield refers to the efficiency of the nonlinear photoemission process. In this database, measurements related to the nonlinear yield of photocathodes will be reported using plots of photocurrent vs incident optical intensity.
Lifetime
Practical photocathode materials need to survive for long amounts of time with limited depredation of their qualities (usually quantum efficiency) in the face of imperfect vacuum conditions. Photocathode lifetime refers to how long a photocathode can survive before degradation occurs. Some factors that can influence this quantity are vacuum poisoning, ion back-bombardment, and exposure to high-intensity laser light. In the database, lifetime is quantified using plots of quantum efficiency versus time or emitted charge.
Three Step Model
Many photocathodes are well described by the "three step" model. Here, the three steps of photoemission (excitation, transport, and escape into vacuum) can be modeled mathematically by the probability distribution of the on-their-way-to-be-emitted electrons at the end of each step. Photocathode properties such as mean transverse energy and quantum efficiency can then be estimated from the final distribution.
If we make some assumptions about the photocathode, these calculations can give especially simple results for key metrics [1]. For a cathode at a temperature of 0 K and a flat band structure, we find that the quantum efficiency changes with the driving laser's excess energy (\(E_{\text{excess}} = h\nu - \phi\), where \(\phi\) is the work function) as \(\text{QE} \propto E_{\text{excess}}^2\) (for small \(E_{\text{excess}}\)) and the mean transverse energy is given by \(\text{MTE}= E_{\text{excess}}/3\). There is a direct tradeoff between trying to minimize the mean transverse energy to get a low emittance electron beam and maximizing the quantum efficiency to get more electrons out (\(\text{QE}\propto(\text{MTE})^2\)).
The following web app displays the momentum distribution of emitted electrons as well as the mean transverse energy. Move the sliders at the top to explore how they change with the laser's excess energy and the photocathode's temperature.
References and Further Reading
- Dowell, D. H., & Schmerge, J. F. (2009). Quantum efficiency and thermal emittance of metal photocathodes. Physical Review Special Topics - Accelerators and Beams, 12(7), 074201.
- Jensen, K. L. (2017). Introduction to the physics of electron emission. John Wiley & Sons.
- Spicer, W. E., & Herrera-Gomez, A. (1993, October). Modern theory and applications of photocathodes. In Photodetectors and Power Meters. SPIE.
- Martinelli, R. U., & Fisher, D. G. (1974). The application of semiconductors with negative electron affinity surfaces to electron emission devices. Proceedings of the IEEE, 62(10), 1339-1360.